Ece OdabaşıT., Gülnur UzunE.
The gene, which is the basic unit of heredity in living things, structurally contains the untranslated region (UTR), enhancer or silencer, and promoter areas at the 5′ and 3′ ends, and coding exons and non-coding introns between them. Gene expression is regulated transcriptionally by messenger RNAs (mRNA) formed as a result of alternative splicing mechanism1. Gene therapy tries to prevent genetic diseases by activating an inactivated gene caused by genetic mutations or by preventing the expression of unwanted genes2. Gene editing technologies provide an entirely new modality for treatments based on precise modification of human genome sequences3,4.
Clustered regulatory spaced short palindromic repeats (CRISPR)/CRISPR-associated protein 9 (Cas9) are novel and proficient RNA-guided endonuclease-based system adapted from the naturally occurring bacterial immune system5 and has been used since 2012 as a genome editing tool as well as gene therapy for the treatment of cancer, infections, and genetic diseases6-9. In this review, future aspects and potential applications of CRISPR/Cas9 technology, which is necessary for the treatment of genetically interacting diseases, will be discussed.
CRISPR/Cas9 system
CRISPR/Cas9 technology, when introduced into a cell, recognizes the conserved sequences of PAM (protospacer adjacent motif) next to the target DNA sequences, single guide RNA (sgRNA), and sgRNA positions the Cas enzyme on the targeted DNA sequence. sgRNA includes trans-activating CRISPR RNA (tracrRNA) and CRISPR RNA (crRNA). TracrRNA binds to the Cas9 protein, which hybridizes with crRNA and forms the CRISPR-Cas9/sgRNA complex to edit genome sequences. Upon this interaction, endonuclease domains cleave both DNA strands three bases upstream of the PAM sequence. The Cas9-sgRNA complex dissolves the double-stranded DNA (dsDNA) so that the complementary sequence in the sgRNA is attached to one of the DNA strands, and Cas nuclease cuts the double strand of DNA, creating a double-strand break (DSB) 10,11. In recent years, the use of CRISPR/Cas strategies, including knock-in (gene insertion, KI), knock-out (gene knockout, KO), base editing (BE), and primary editing (PE) in variations that cause genetic diseases, has brought a groundbreaking view to gene therapy 2,12(Figure 1).
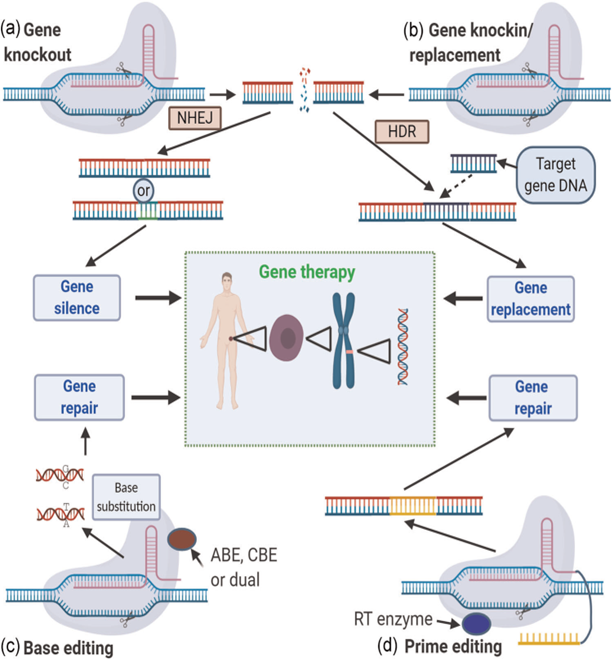
Figure 1. An overview of the four main techniques of CRISPR/Cas gene therapy2. a) Gene knock out. b) Gene knock-in/replacement. c) Base arrangement d) Primary arrangement.
1. DSB-mediated Gene Editing
There are many coding and non-coding genes in organisms. Of the non-coding genes, most of the long noncoding RNA (long ncRNA) and microRNA (miRNA), known as genes that play a role in cell physiology and regulate gene expression, serve as tumor suppressors and proto-oncogenes13,14. The KO method involves introducing CRISPR/Cas9 into the cell for genetic manipulation, and various repair mechanisms in the cell are activated after DSBs are formed in the DNA. In the non-homologous end-joining (NHEJ) mechanism, protein factors recombine directly or by incorporating nucleotide deletions or insertions into DNA strands; however, the open reading frame (ORF) of the gene is changing, so it is possible to silence or delete an unwanted gene by creating KO15,16.
A repair mechanism called Homology Directed Recombination (HDR) is used in the KI method. It differs from NHEJ in that it requires a template to repair DSBs, which can be endogenous or exogenous, inserted into its genome, or composed of homologous DNA sequences. Duplication occurs when cells use homologous sequences as DNA templates. Exogenous DNA templates, on the other hand, cause horizontal gene transfer so that an undesired region of the genome can be converted into a determined gene sequence17. NHEJ and HDR mechanisms often result in small insertions/deletions (indels), out-of-frame mutations, early stop codons, or loss of function18,19. The low success frequency of KO and KI methods led to the development of BE and PE methods17.
2. Base Editing
Mutations called single nucleotide polymorphism (SNP) resulting from a single base change constitute the proportionally largest proportion of human genetic diseases20. Base editing is an approach based on irreversibly changing one base pair to another base pair at the genomic target locus and does not require DSB, HDR, or donor DNA21. In this way, errors that occur during DNA repair are minimized. DNA base modifiers are formed by a catalytically inactivated Cas nuclease enzyme (dead Cas9/dCas9) or a deaminase fused nickase Cas9 (nCas9). After cleavage at the target locus, a deaminase binds to the opposite DNA strand and modifies the bases. Base editing tools21 (Figure 2), which are divided into two classes as cytosine base editors (cytosine base editor, CBE)22 and adenine base editors (ABE)23, are frequently used as they have a relatively low-risk ratio compared to CRISPR-Cas systems inducing DSBs, although they show indel risk24,25.
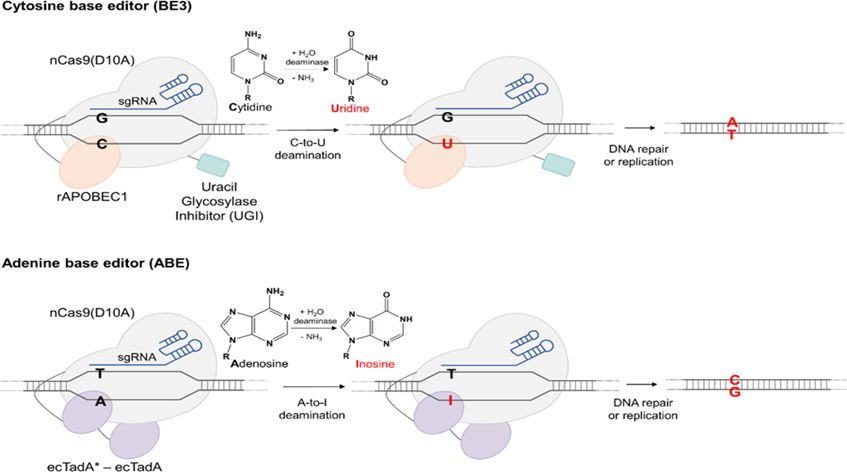
Figure 2. Cytosine Base Regulator (CBE) and Adenine Base Regulator (ABE)21. CBE is able to bind to target DNA under the direction of Cas9. Apoliprotein mRNA cytidine deaminase 1 (APOBEC1) catalyzes the hydrolytic deamination of cytosine (C) in DNA and RNA to form uracil (U). It is then converted to thymine (T) by DNA repair mechanisms. When Cas9 directs ABE to the desired region in the target DNA, adenine (A) is converted to inosine (I) by adenine deaminase (ecTadA). It is converted to guanine (G) by DNA repair mechanisms.
2.1. Cytosine Base Regulators (CBE)
It mainly consists of CBEs, Cas nuclease, and activation-induced cytidine deaminase, AID (APOBEC/AID). Advanced top models have been designed since the first base editor (BE1), which was developed by combining APOBEC1 with the N-terminus of the dCas9 nuclease26. The BE-sgRNA complex binds to the target genomic DNA. This results in an RNA-DNA hybrid and a single-stranded DNA loop not bound to sgRNA around the target site. ssDNA can then be used for rAPOBEC1-catalyzed deamination of cytosine within the five nucleotide window. The second developed cytosine base regulator (CBE2) was developed by the fusion of the uracil DNA glycosylase inhibitor (UGI) with BE1. CBE3 is formed by the recovery of the histidine amino acid (H840, HNH catalytic domain) at position 840 in dCas923-25.
2.2. Adenine Base Regulators (ABE)
For the first ABE designed with theantibiotic resistance complement approach, TadA mutants, and defective antibiotic resistance genes were generated in Escherichia coli cells. TadA-dCas9 fusion corrected the targeted A base in the mutant chloramphenicol resistance gene. ABE1 is XTEN to the N-terminus of nCas9 in the TadA variant (TadA*) (16 amino acids linked are used in BE3); It was formed by the addition of the nuclear localization signal (NLS) to the C terminal (TadA*-XTEN-nCas9-NLS). It can change to A-G or A-T to G-C by adenosine deamination27.
3. Prime Editing
PE, developed by Anzalone et al., is a gene editing method that can perform targeted small indels and base changes, does not cause DSBs that occur in classical CRISPR methods, and does not require a donor DNA template28,29. PEs use a primary editing guide RNA (pegRNA) hybridized to the target DNA region and a nCas9-fused reverse transcriptase (RT) enzyme30. The 5′ end of the pegRNA binds to the primer binding sequence (PBS) of the DNA, showing the non-complementary sequence. The PAM-containing chain is nicked by Cas9 and forms a primer for nCas9-dependent RT. Thanks to RT, the nicked PAM sequence uses the interior of the pegRNA as a template and makes the target region programmable (Figure 3) 31. With nCas9, targeted indels and off-target effects can be predicted in the genome. PE has the ability to regulate target frameshift or indel mutations with much higher efficiency than HDR32. Three major regulatory (PE) systems have been established and tested in human cells. The first system (PE1) was constructed with a fusion of Cas9(H840A) nicase and wild-type Moloney murine leukemia virus RT enzyme. In the second system (PE2), five specific mutations were added to M-MLV RT to increase the stability, affinity, and processing of DNA-RNA substrates28.
While L603W, D200N, and 330P mutations increase the number of transversions applied at high temperatures and RT activity, RT thermostability and the bond of the RT-template-PBS complex are strengthened thanks to two mutations called W313F and T306K. The PE2 system consists of the fusion of pentamutant RT with nCas9. PE2 showed a 5.1-fold improvement in the efficiency of the main regulation point mutation compared to PE133. The PE3 system differs from PE2 in that it cuts the unedited strand away from the pegRNA target and uses an additional gRNA (sgRNA) for this. Thus, regulation efficiency increases34,35.
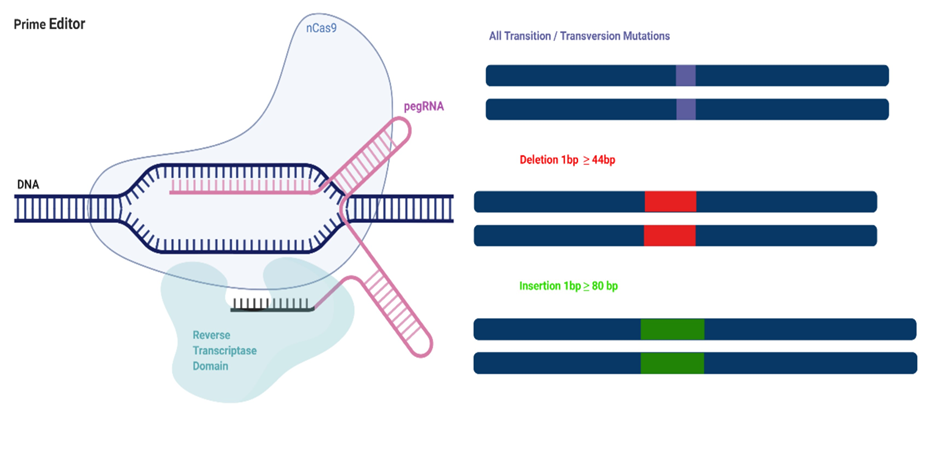
Figure 3. Primary Arrangement31. PEs consist of an nCas9 fused reverse transcriptase (RT) and a pegRNA. The pegRNA has two sequences that direct nCas9 to the target sequence and contain the desired changes. nCas9 nicks the PAM sequence after binding to PBS. Thanks to PE, all transversion/transition mutations and small indels can be edited.
4. Primary Regulation and Base Regulation Application Areas
PEs have been tested in post-mitotic mouse cortical neurons, multiple human cell lines29, mouse embryos36, human pluripotent stem cells (iPSC)37, rice and wheat protoplasts30,38-40 and supported with varying efficiencies. In short, it has been shown that in DGAT1 (Diacylglycerol O-acyltransferase 1) deficiency in Wilson’s disease, which is known as copper accumulation in the organs, the in-frame deletions and frameshift mutations that cause the disease are corrected by PE41. Rousseau et al., using PE, aimed to prevent the development of the disease by creating the Ala673Thr variant in the APP gene, which has an important role in Alzheimer’s disease42. Thanks to BE, fetal hemoglobin (alpha2gamma2) of transcription factor B-cell lymphoma/leukemia 11A (BCL11A) was suppressed in adult-stage erythroid cells, hereby treating the core sequence β-thalassemia-induced disorder41. The disease phenotype was corrected by transforming GGT>CCC in the β-thalassemia mouse model with IVS-II-654 mutation with PE343. Krishnamurthy et al. developed a tool to repair rare cystic fibrosis transmembrane conductance regulator (CFTR) mutations by delivering BE ribonucleoproteins (RNP) to human airway epithelial cells; thus, a viable gene editing strategy for cystic fibrosis disease has been demonstrated44. CRISPR/Cas9 technology holds great promise as a treatment for existing chronic viral infections such as human immunodeficiency virus (HIV), human papillomavirus (HPV), hepatitis B virus (HBV), and herpesvirus45-47. Studies on CRISPR–Cas9 applications have also been conducted in different types of cancer treatment48-53. Today, important experimental studies are carried out on monogenic diseases54-57 and antibiotic resistance in model organisms58.
In conclusion, CRISPR-Cas-based gene therapy has been a promising approach for the treatment of genetic diseases. CRISPR-Cas9 technology has been used in a wide range of domains, from fundamental biology to cancer therapy, since it is a potent, low-cost, and easy-to-create genome editing tool. Research on the use of this system in the treatment of various diseases continues rapidly.
Reference:
- Kumar, A., & Bansal, M. (2018). Modulation of Gene Expression by Gene Architecture and Promoter Structure. Bioinformatics in the Era of Post Genomics and Big Data. https://doi.org/10.5772/INTECHOPEN.76051
- Zhang, B. (2021). CRISPR/Cas gene therapy. Journal of Cellular Physiology, 236(4), 2459–2481. https://doi.org/10.1002/JCP.30064
- Mullard, A. (2020). Gene-editing pipeline takes off. Nature Reviews. Drug Discovery, 19(6), 367–372. https://doi.org/10.1038/D41573-020-00096-Y
- Finn, J. D., Smith, A. R., Patel, M. C., Shaw, L., Youniss, M. R., van Heteren, J., Dirstine, T., Ciullo, C., Lescarbeau, R., Seitzer, J., Shah, R. R., Shah, A., Ling, D., Growe, J., Pink, M., Rohde, E., Wood, K. M., Salomon, W. E., Harrington, W. F., … Morrissey, D. V. (2018). A Single Administration of CRISPR/Cas9 Lipid Nanoparticles Achieves Robust and Persistent In Vivo Genome Editing. Cell Reports, 22(9), 2227–2235. https://doi.org/10.1016/J.CELREP.2018.02.014
- Young, C. S., Pyle, A. D., & Spencer, M. J. (2019). CRISPR for Neuromuscular Disorders: Gene Editing and Beyond. Physiology (Bethesda, Md.), 34(5), 341–353. https://doi.org/10.1152/PHYSIOL.00012.2019
- Gasiunas, G., Barrangou, R., Horvath, P., & Siksnys, V. (2012). Cas9-crRNA ribonucleoprotein complex mediates specific DNA cleavage for adaptive immunity in bacteria. Proceedings of the National Academy of Sciences of the United States of America, 109(39), E2579–E2586. https://doi.org/10.1073/PNAS.1208507109/SUPPL_FILE/PNAS.201208507SI.PDF
- Jinek, M., Chylinski, K., Fonfara, I., Hauer, M., Doudna, J. A., & Charpentier, E. (2012). A programmable dual-RNA-guided DNA endonuclease in adaptive bacterial immunity. Science, 337(6096), 816–821. https://doi.org/10.1126/SCIENCE.1225829/SUPPL_FILE/JINEK.SM.PDF
- Doudna, J. A., & Charpentier, E. (2014). The new frontier of genome engineering with CRISPR-Cas9. Science, 346(6213). https://doi.org/10.1126/SCIENCE.1258096
- Lee, M. H., Shin, J. Il, Yang, J. W., Lee, K. H., Cha, D. H., Hong, J. B., Park, Y., Choi, E., Tizaoui, K., Koyanagi, A., Jacob, L., Park, S., Kim, J. H., & Smith, L. (2022). Genome Editing Using CRISPR-Cas9 and Autoimmune Diseases: A Comprehensive Review. International Journal of Molecular Sciences, 23(3). https://doi.org/10.3390/IJMS23031337
- Zhang, D., Hussain, A., Manghwar, H., Xie, K., Xie, S., Zhao, S., Larkin, R. M., Qing, P., Jin, S., & Ding, F. (2020). Genome editing with the CRISPR-Cas system: an art, ethics and global regulatory perspective. Plant Biotechnology Journal, 18(8), 1651–1669. https://doi.org/10.1111/PBI.13383
- Nidhi, S., Anand, U., Oleksak, P., Tripathi, P., Lal, J. A., Thomas, G., Kuca, K., & Tripathi, V. (2021). Novel CRISPR–Cas Systems: An Updated Review of the Current Achievements, Applications, and Future Research Perspectives. International Journal of Molecular Sciences, 22(7). https://doi.org/10.3390/IJMS22073327
- Thomson, M. J., Biswas, S., Tsakirpaloglou, N., & Septiningsih, E. M. (2022). Functional Allele Validation by Gene Editing to Leverage the Wealth of Genetic Resources for Crop Improvement. International Journal of Molecular Sciences 2022, Vol. 23, Page 6565, 23(12), 6565. https://doi.org/10.3390/IJMS23126565
- Fu, Y. W., Dai, X. Y., Wang, W. T., Yang, Z. X., Zhao, J. J., Zhang, J. P., Wen, W., Zhang, F., Oberg, K. C., Zhang, L., Cheng, T., & Zhang, X. B. (2021). Dynamics and competition of CRISPR–Cas9 ribonucleoproteins and AAV donor-mediated NHEJ, MMEJ and HDR editing. Nucleic Acids Research, 49(2), 969–985. https://doi.org/10.1093/NAR/GKAA1251
- Panni, S., Lovering, R. C., Porras, P., & Orchard, S. (2020). Non-coding RNA regulatory networks. Biochimica et Biophysica Acta (BBA) – Gene Regulatory Mechanisms, 1863(6), 194417. https://doi.org/10.1016/J.BBAGRM.2019.194417
- Makarova, K. S., Wolf, Y. I., Iranzo, J., Shmakov, S. A., Alkhnbashi, O. S., Brouns, S. J. J., Charpentier, E., Cheng, D., Haft, D. H., Horvath, P., Moineau, S., Mojica, F. J. M., Scott, D., Shah, S. A., Siksnys, V., Terns, M. P., Venclovas, Č., White, M. F., Yakunin, A. F., … Koonin, E. V. (2019). Evolutionary classification of CRISPR–Cas systems: a burst of class 2 and derived variants. Nature Reviews Microbiology 2019 18:2, 18(2), 67–83. https://doi.org/10.1038/s41579-019-0299-x
- Lino, C. A., Harper, J. C., Carney, J. P., & Timlin, J. A. (2018). Delivering CRISPR: a review of the challenges and approaches. Drug Delivery, 25(1), 1234. https://doi.org/10.1080/10717544.2018.1474964
- Devkota, S. (2018). The road less traveled: strategies to enhance the frequency of homology-directed repair (HDR) for increased efficiency of CRISPR/Cas-mediated transgenesis. BMB Reports, 51(9), 437–443. https://doi.org/10.5483/BMBREP.2018.51.9.187
- Jiang, H., Jing, Q., Yang, Q., Qiao, C., Liao, Y., Liu, W., & Xing, Y. (2022). Efficient Simultaneous Introduction of Premature Stop Codons in Three Tumor Suppressor Genes in PFFs via a Cytosine Base Editor. Genes, 13(5), 835. https://doi.org/10.3390/GENES13050835/S1
- Ishibashi, A., Saga, K., Hisatomi, Y., Li, Y., Kaneda, Y., & Nimura, K. (2020). A simple method using CRISPR-Cas9 to knock-out genes in murine cancerous cell lines. Scientific Reports 2020 10:1, 10(1), 1–10. https://doi.org/10.1038/s41598-020-79303-0
- Goodwin, S., McPherson, J. D., & McCombie, W. R. (2016). Coming of age: ten years of next-generation sequencing technologies. Nature Reviews Genetics 2016 17:6, 17(6), 333–351. https://doi.org/10.1038/nrg.2016.49
- Park, S., & Beal, P. A. (2019). Off-Target Editing by CRISPR-Guided DNA Base Editors. Biochemistry, 58(36), 3727–3734. https://doi.org/10.1021/ACS.BIOCHEM.9B00573/ASSET/IMAGES/MEDIUM/BI9B00573_0005.GIF
- Komor, A. C., Kim, Y. B., Packer, M. S., Zuris, J. A., & Liu, D. R. (2016). Programmable editing of a target base in genomic DNA without double-stranded DNA cleavage. Nature 2015 533:7603, 533(7603), 420–424. https://doi.org/10.1038/nature17946
- Gaudelli, N. M., Komor, A. C., Rees, H. A., Packer, M. S., Badran, A. H., Bryson, D. I., & Liu, D. R. (2017). Programmable base editing of A•T to G•C in genomic DNA without DNA cleavage. Nature 2017 551:7681, 551(7681), 464–471. https://doi.org/10.1038/nature24644
- Rees, H. A., & Liu, D. R. (2018). Base editing: precision chemistry on the genome and transcriptome of living cells. Nature Reviews Genetics 2018 19:12, 19(12), 770–788. https://doi.org/10.1038/s41576-018-0059-1
- Kurt, I. C., Zhou, R., Iyer, S., Garcia, S. P., Miller, B. R., Langner, L. M., Grünewald, J., & Joung, J. K. (2020). CRISPR C-to-G base editors for inducing targeted DNA transversions in human cells. Nature Biotechnology 2020 39:1, 39(1), 41–46. https://doi.org/10.1038/s41587-020-0609-x
- Porto, E. M., Komor, A. C., Slaymaker, I. M., & Yeo, G. W. (2020). Base editing: advances and therapeutic opportunities. Nature Reviews. Drug Discovery, 19(12), 839. https://doi.org/10.1038/S41573-020-0084-6
- Eid, A., Alshareef, S., & Mahfouz, M. M. (2018). CRISPR base editors: genome editing without double-stranded breaks. Biochemical Journal, 475(11), 1955–1964. https://doi.org/10.1042/BCJ20170793
- Anzalone, A. V., Randolph, P. B., Davis, J. R., Sousa, A. A., Koblan, L. W., Levy, J. M., Chen, P. J., Wilson, C., Newby, G. A., Raguram, A., & Liu, D. R. (2019). Search-and-replace genome editing without double-strand breaks ordonor DNA. Nature, 576(7785), 149. https://doi.org/10.1038/S41586-019-1711-4
- Marzec, M., Brąszewska-Zalewska, A., & Hensel, G. (2020). Prime Editing: A New Way for Genome Editing. Trends in Cell Biology, 30(4), 257–259. https://doi.org/10.1016/J.TCB.2020.01.004
- Xu, W., Zhang, C., Yang, Y., Zhao, S., Kang, G., He, X., Song, J., & Yang, J. (2020). Versatile Nucleotides Substitution in Plant Using an Improved Prime Editing System. Molecular Plant, 13(5), 675–678. https://doi.org/10.1016/j.molp.2020.03.012
- Kantor, A., McClements, M. E., & Maclaren, R. E. (2020). CRISPR-Cas9 DNA Base-Editing and Prime-Editing. International Journal of Molecular Sciences 2020, Vol. 21, Page 6240, 21(17), 6240. https://doi.org/10.3390/IJMS21176240
- Scholefield, J., & Harrison, P. T. (2021). Prime editing – an update on the field. Gene Therapy 2021 28:7, 28(7), 396–401. https://doi.org/10.1038/s41434-021-00263-9
- Jiang, Z., Abdullah, Zhang, S., Jiang, Y., Liu, R., & Xiao, Y. (2022). Development and Optimization of CRISPR Prime Editing System in Photoautotrophic Cells. Molecules, 27(6), 1758. https://doi.org/10.3390/MOLECULES27061758/S1
- Anzalone, A. V., Koblan, L. W., & Liu, D. R. (2020). Genome editing with CRISPR–Cas nucleases, base editors, transposases and prime editors. Nature Biotechnology 2020 38:7, 38(7), 824–844. https://doi.org/10.1038/s41587-020-0561-9
- Chen, P. J., Hussmann, J. A., Yan, J., Knipping, F., Ravisankar, P., Chen, P. F., Chen, C., Nelson, J. W., Newby, G. A., Sahin, M., Osborn, M. J., Weissman, J. S., Adamson, B., & Liu, D. R. (2021). Enhanced prime editing systems by manipulating cellular determinants of editing outcomes. Cell, 184(22), 5635-5652.e29. https://doi.org/10.1016/j.cell.2021.09.018
- Liu, Y., Li, X., He, S., Huang, S., Li, C., Chen, Y., Liu, Z., Huang, X., & Wang, X. (2020). Efficient generation of mouse models with the prime editing system. Cell Discovery 2020 6:1, 6(1), 1–4. https://doi.org/10.1038/s41421-020-0165-z
- Sürün, D., Schneider, A., Mircetic, J., Neumann, K., Lansing, F., Paszkowskirogacz, M., Hänchen, V., Leekirsch, M. A., & Buchholz, F. (2020). Efficient Generation and Correction of Mutations in Human iPS Cells Utilizing mRNAs of CRISPR Base Editors and Prime Editors. Genes 2020, Vol. 11, Page 511, 11(5), 511. https://doi.org/10.3390/GENES11050511
- Lin, Q., Zong, Y., Xue, C., Wang, S., Jin, S., Zhu, Z., Wang, Y., Anzalone, A. V., Raguram, A., Doman, J. L., Liu, D. R., & Gao, C. (2020). Prime genome editing in rice and wheat. Nature Biotechnology 2020 38:5, 38(5), 582–585. https://doi.org/10.1038/s41587-020-0455-x
- Tang, X., Sretenovic, S., Ren, Q., Jia, X., Li, M., Fan, T., Yin, D., Xiang, S., Guo, Y., Liu, L., Zheng, X., Qi, Y., & Zhang, Y. (2020). Plant Prime Editors Enable Precise Gene Editing in Rice Cells. Molecular Plant, 13(5), 667–670. https://doi.org/10.1016/j.molp.2020.03.010
- Li, H., Li, J., Chen, J., Yan, L., & Xia, L. (2020). Precise Modifications of Both Exogenous and Endogenous Genes in Rice by Prime Editing. Molecular Plant, 13(5), 671–674. https://doi.org/10.1016/j.molp.2020.03.011
- Antoniou, P., Miccio, A., & Brusson, M. (2021). Base and Prime Editing Technologies for Blood Disorders. Frontiers in Genome Editing, 0, 1. https://doi.org/10.3389/FGEED.2021.618406
- Rousseau, J., Mbakam, C. H., Guyon, A., Tremblay, G., Begin, F. G., & Tremblay, J. P. (2020). Specific mutations in genes responsible for Alzheimer and for Duchenne Muscular Dystrophy introduced by Base editing and PRIME editing. BioRxiv, 2020.07.31.230565. https://doi.org/10.1101/2020.07.31.230565
- Zhang, H., Sun, R., Fei, J., Chen, H., & Lu, D. (2022). Correction of Beta-Thalassemia IVS-II-654 Mutation in a Mouse Model Using Prime Editing. International Journal of Molecular Sciences 2022, Vol. 23, Page 5948, 23(11), 5948. https://doi.org/10.3390/IJMS23115948
- Krishnamurthy, S., Traore, S., Cooney, A. L., Brommel, C. M., Kulhankova, K., Sinn, P. L., Newby, G. A., Liu, D. R., & McCray, P. B. (2021). Functional correction of CFTR mutations in human airway epithelial cells using adenine base editors. Nucleic Acids Research, 49(18), 10558–10572. https://doi.org/10.1093/NAR/GKAB788
- Lee, C. (2019). CRISPR/Cas9-Based Antiviral Strategy: Current Status and the Potential Challenge. Molecules, 24(7). https://doi.org/10.3390/MOLECULES24071349
- Zhen, S., Hua, L., Liu, Y. H., Gao, L. C., Fu, J., Wan, D. Y., Dong, L. H., Song, H. F., & Gao, X. (2015). Harnessing the clustered regularly interspaced short palindromic repeat (CRISPR)/CRISPR-associated Cas9 system to disrupt the hepatitis B virus. Gene Therapy, 22(5), 404–412. https://doi.org/10.1038/GT.2015.2
- Kaminski, R., Chen, Y., Fischer, T., Tedaldi, E., Napoli, A., Zhang, Y., Karn, J., Hu, W., & Khalili, K. (2016). Elimination of HIV-1 Genomes from Human T-lymphoid Cells by CRISPR/Cas9 Gene Editing. Scientific Reports, 6. https://doi.org/10.1038/SREP22555
- Cheung, A. H. K., Chow, C., Zhang, J., Zhou, Y., Huang, T., Ng, K. C. K., Or, T. C. T., Yao, Y. Y., Dong, Y., Fung, J. M. W., Xiong, L., Chan, A. K. Y., Lung, W. M. R., Kang, W., & To, K. F. (2018). Specific targeting of point mutations in EGFR L858R-positive lung cancer by CRISPR/Cas9. Laboratory Investigation; a Journal of Technical Methods and Pathology, 98(7), 968–976. https://doi.org/10.1038/S41374-018-0056-1
- Mintz, R. L., Lao, Y. H., Chi, C. W., He, S., Li, M., Quek, C. H., Shao, D., Chen, B., Han, J., Wang, S., & Leong, K. W. (2020). CRISPR/Cas9‐mediated mutagenesis to validate the synergy between PARP1 inhibition and chemotherapy in BRCA1‐mutated breast cancer cells. Bioengineering & Translational Medicine, 5(1). https://doi.org/10.1002/BTM2.10152
- Almeida, R. M. de, Bandarra, S., Ribeiro, A. C., Mascarenhas, P., Bekman, E., & Barahona, I. (2019). Inactivation of APOBEC3G gene in breast cancer cells using the CRISPR/Cas9 system. Https://Doi.Org/10.1080/07853890.2018.1561848, 51(sup1), 40–40. https://doi.org/10.1080/07853890.2018.1561848
- Xue, W., Chen, S., Yin, H., Tammela, T., Papagiannakopoulos, T., Joshi, N. S., Cai, W., Yang, G., Bronson, R., Crowley, D. G., Zhang, F., Anderson, D. G., Sharp, P. A., & Jacks, T. (2014). CRISPR-mediated direct mutation of cancer genes in the mouse liver. Nature, 514(7522), 380. https://doi.org/10.1038/NATURE13589
- Wan, T., Chen, Y., Pan, Q., Xu, X., Kang, Y., Gao, X., Huang, F., Wu, C., & Ping, Y. (2020). Genome editing of mutant KRAS through supramolecular polymer-mediated delivery of Cas9 ribonucleoprotein for colorectal cancer therapy. Journal of Controlled Release : Official Journal of the Controlled Release Society, 322, 236–247. https://doi.org/10.1016/J.JCONREL.2020.03.015
- Batır, M. B., Şahin, E., & Çam, F. S. (2019). Evaluation of the CRISPR/Cas9 directed mutant TP53 gene repairing effect in human prostate cancer cell line PC-3. Molecular Biology Reports, 46(6), 6471–6484. https://doi.org/10.1007/S11033-019-05093-Y
- Maeder, M. L., Stefanidakis, M., Wilson, C. J., Baral, R., Barrera, L. A., Bounoutas, G. S., Bumcrot, D., Chao, H., Ciulla, D. M., DaSilva, J. A., Dass, A., Dhanapal, V., Fennell, T. J., Friedland, A. E., Giannoukos, G., Gloskowski, S. W., Glucksmann, A., Gotta, G. M., Jayaram, H., … Jiang, H. (2019). Development of a gene-editing approach to restore vision loss in Leber congenital amaurosis type 10. Nature Medicine, 25(2), 229–233. https://doi.org/10.1038/S41591-018-0327-9
- Nelson, C. E., Hakim, C. H., Ousterout, D. G., Thakore, P. I., Moreb, E. A., Castellanos Rivera, R. M., Madhavan, S., Pan, X., Ran, F. A., Yan, W. X., Asokan, A., Zhang, F., Duan, D., & Gersbach, C. A. (2016). In vivo genome editing improves muscle function in a mouse model of Duchenne muscular dystrophy. Science (New York, N.Y.), 351(6271), 403. https://doi.org/10.1126/SCIENCE.AAD5143
- Wu, Y., Zeng, J., Roscoe, B. P., Liu, P., Yao, Q., Lazzarotto, C. R., Clement, K., Cole, M. A., Luk, K., Baricordi, C., Shen, A. H., Ren, C., Esrick, E. B., Manis, J. P., Dorfman, D. M., Williams, D. A., Biffi, A., Brugnara, C., Biasco, L., … Bauer, D. E. (2019). Highly efficient therapeutic gene editing of human hematopoietic stem cells. Nature Medicine, 25(5), 776. https://doi.org/10.1038/S41591-019-0401-Y
- György, B., Nist-Lund, C., Pan, B., Asai, Y., Karavitaki, K. D., Kleinstiver, B. P., Garcia, S. P., Zaborowski, M. P., Solanes, P., Spataro, S., Schneider, B. L., Joung, J. K., Géléoc, G. S. G., Holt, J. R., & Corey, D. P. (2019). Allele-specific gene editing prevents deafness in a model of dominant progressive hearing loss. Nature Medicine, 25(7), 1123. https://doi.org/10.1038/S41591-019-0500-9
- Tao, S., Chen, H., Li, N., & Liang, W. (2022). The Application of the CRISPR-Cas System in Antibiotic Resistance. Infection and Drug Resistance, 15, 4155. https://doi.org/10.2147/IDR.S370869