Sarehan AkyüzT, Ebru KavaklıE.
Monitoring of chemical and biological processes is of great importance in the field of medical and biological applications1. Dynamic and real-time monitoring of these processes involves complex steps, and innovations in this field provide optimization and control of the raw materials2. Analyzing quantitative and qualitative data using conventional analytical methods can be time-consuming and economically challenging. Biosensors provide a crucial opportunity for real-time monitoring in the analysis of these complex data, compared to traditional methods that complicate in vivo applications3.
Biosensors are commonly described as analytical devices that convert biological responses into electrical signals4. Biosensors consist of an analyte (bio descriptor element), a bioreceptor, a transducer, a signal processor that converts electrical signals into target signals, and interface components (Figure 1)5. Developing a biosensor requires defining the target analyte, selecting the bioreceptor according to the obtained data, and completing it with the appropriate transducer. In an ideal biosensor design, parameters such as high selectivity, accuracy, stability, and a wide evaluation range are essential6. The analyte, as one of the components, enables the application of the biocatalytic process or mediates the specific interactions that the analyte will carry out7. Biocatalytic receptors can include enzymes, cells, cell organelles, tissues, or even whole organisms in their structure. Bioreceptors, which are in the form of biological macromolecules, transmit signals they generate to the transducer component in accordance with their binding function to the biological sample or the analyte surface6. The interactions between analytes and bioreceptors can result in differences in the scope of light, heat, pH change, mass change, or charge value change6. Biosensors are classified based on different material forms or transduction mechanisms used by the sensor, and as a second classification category, they are classified according to the biological recognition element (analyte)8. Electrochemical, optical, piezoelectric, mass-based, thermal, or ion-selective electrodes are examples of the classification based on the transduction mechanism. The classification based on the analyte includes nucleic acids, aptamer (peptide molecules), cell, biomolecules, biomimetic, and enzyme-based systems9.
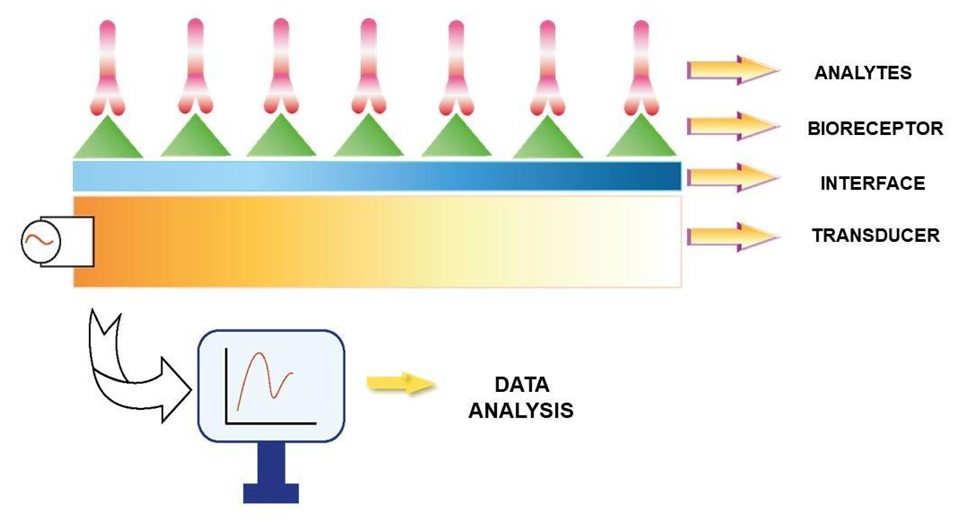
Figure 1. The schematic design and mechanism of the bioreceptor components10. The components of the biosensor structure, including the analyte, bioreceptor, interface, and transducer elements are represented using a basic design model.
Electrochemical biosensors are devices that convert biochemical events into electrical signals. Electrochemical transducers detect the presence of ions/electrons generated or utilized by the interaction between the target analyte and the biomolecule11,12. The amperometric method is used to measure the flow between electrodes in redox reactions. The operating principle of amperometric biosensors is based on the potential of electro-activity value between electrodes resulting from oxidation or reduction reactions. Potentiometric biosensors assess pH variations and ion concentration brought on by interactions between antigen and antibody.13. The working principle is based on the occurrence of the current flow in parallel to the chemical reactions when voltage is applied to the electrode in the solution. Due to its basic structure and sensitive detection mechanism, the impedimetric method is preferred for preventing food hazards14,15,16.
Optical biosensors are highly effective in biological systems, clinical diagnosis, drug discovery, and control stages for food and environmental observations17. These biosensors provide durability, stability, and the advantage of being integrated into a chip, in addition to their highly sensitive measurement potential. Optical biosensors perform quality assessment on treatments that include antibiotics, anticonvulsants (the main drug group for epilepsy), and anti-cancer drugs18. This feature has positive effects on therapeutic drug monitoring (TDM), which is one of the fundamental methods used to minimize the highly toxic effects and dosage administration that poses a risk for patients undergoing treatment18.
Piezoelectric crystals exist in the structure of piezoelectric biosensors, which include analytic devices based on affinity interactions19. The mechanism that operates based on the crystal surface area and mass change is referred to as the ability of piezoelectric material to generate voltage due to mechanical stress19,20. The mechanism can work oppositely when the voltage is variable and generates mechanical stress or vibrations. After this stage, crystals dependent on the molecule or component gain a new value by not creating their characteristic frequency echo21.
Mass-based (gravimetric) biosensors operate based on the response system to mass changes. In the structure of biosensors, quartz crystal microbalance (QCM), a form of piezoelectric crystals, or surface acoustic wave (SAW) devices are mostly used22. QCM enables the detection of pathogens and toxic substances, while the sensitive coating material on the sensor surface allows for the environmental detection of the target analyte13,22. Thermal (calorimetric) biosensors, scale the concentration of the target analyte based on its production or fading in biochemical reactions. The scaled energy source changes in direct proportion to the number of molecules generated in the biochemical reaction22. Nucleic acid-based structures, one of the biosensor types categorized under the analyte heading, include deoxyribonucleic acid (DNA), ribonucleic acid (RNA), peptide nucleic acid (PNA), and designs generated across aptamers23. The working principle of nucleic acid-based biosensors relies on the hybridization mechanism. This method relies on the immobilization of the sample DNA sequence on the transducer and the subsequent creation of complementary sequences parallel to the incomplete sequence. DNA-based biosensors are composed of genetic materials in the form of single-stranded DNA (ssDNA), double- stranded DNA (dsDNA) circular DNA, and triple-stranded (triplex) DNA23. They enable biomedical-based detections through their strong chemical structures and bio-sensing functions23,24 (Figure 2)23. The principles of sensors that use DNA technology rely on the binding capacity of DNA nanostructures to target analytes; the differences that occur in the nanoarchitecture of DNA upon binding are utilized for reading the signal value25.
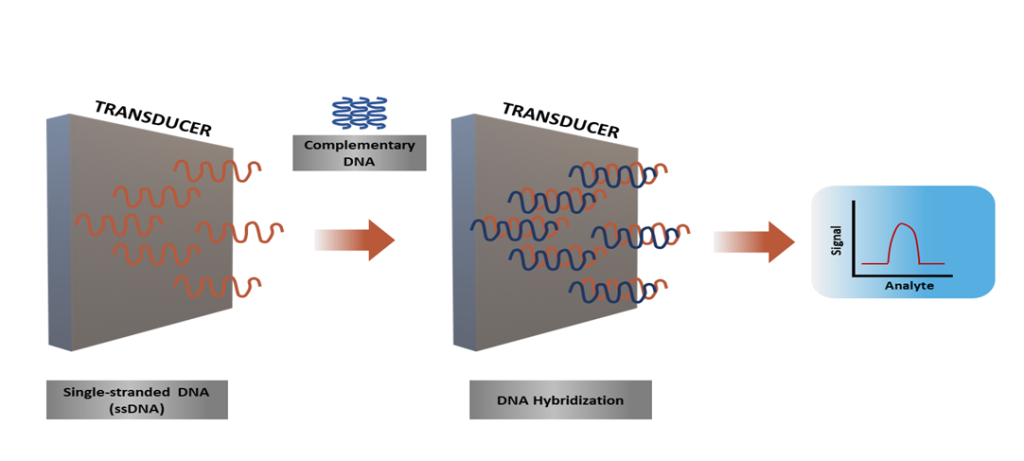
Figure 2. The working mechanism of nucleic acid-based biosensors23. The biorecognition step in nucleic acid-based biosensors used to detect nucleic acids involves the pairing process between the DNA probe and the target strands, which is known as hybridization.
RNA-based biosensors play a critical role in gene regulation as an efficient and minimally invasive biomarker component26. They are involved in gene expression and regulation as equivalents in physiological functions to messenger RNA (mRNA), microRNA (miRNA), and long non-coding RNA (lncRNA)26. Because of these effects, they are important in diagnosing diseases and determining the appropriate treatment methods26.
Biomimetic biosensors are structures that have the ability to mimic the functions of natural biosensors. Aptasensors, one of its subcategories, utilize aptamers as bio-complementary agents to mimic chemical structures and structural similarities, resulting in more effective methods27. Aptasensors consist of ssDNA and RNA nucleic acid sequences that bind to proteins, small molecules (micromolecules), metal ions, bacterial cells, viruses, cancer cells, and target molecules exemplified by tissue with high selectivity. Aptasensors are used within the scope of disease detection, drug delivery, cellular imaging in therapeutics, bio-sensing, and biomarkers28,29. Additionally, aptamer-based systems are being developed to identify environmental contaminants in water sources, including heavy metals, agricultural poisons, and bacterial pathogens 30.
Immunosensors detect the specific bond between antibodies and antigen targets and form a stable immune complex structure31. This complex structure enables the identification of molecules between antigens/antibodies due to the binding of immunochemical reactions to the transducer32. Due to the rapid course of identifying specific antigens, controlling the reactions is brought to a more reliable level33. Also, adding an antibody combined with a marker to the system enables the recognition of primary antigens or antibodies32,33,34. The immobilization of enzymes is uniquely applied in enzyme-based biosensors based on the active renewal data of enzymes35. The most common methods for immobilization of enzymes are absorption methods involving weak bonds, electrostatic forces, or hydrophobic interactions. The use of immobilized enzymes is significantly essential in food control and biomedical applications35.
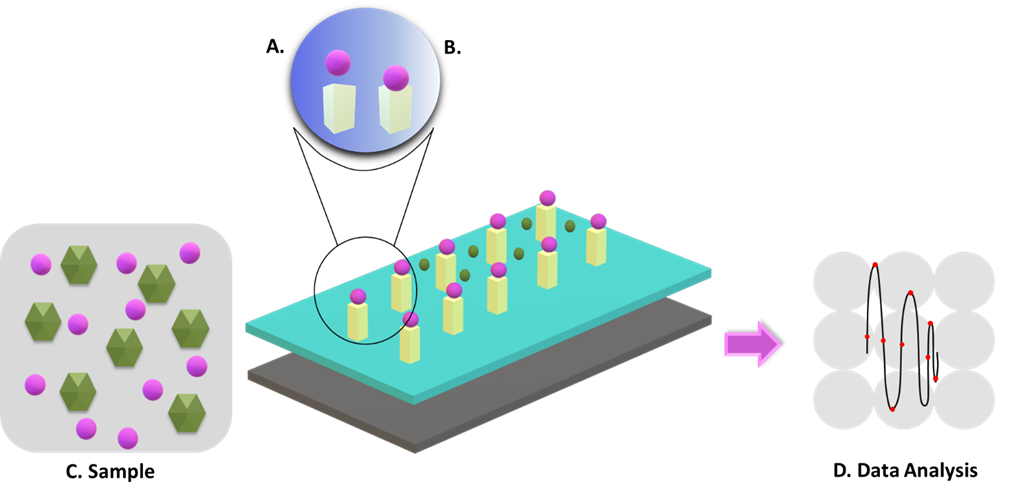
Figure 3. Architecture of biosensors 36. The target analyte is detected by the bioreceptor, and the resulting signals are transmitted to the transducer for data analysis. (A) Biosensor electrode: consists of bioreceptor immobilizer surface and transducer; (B) Physicochemical reaction; (C) Sample; (D) Data Analysis
Today, biosensors are widely used, especially in clinical treatments, pharmacy, biomedical, and healthcare services. Additionally, monitoring the natural environment, conducting molecular diagnoses, detecting pathogens, and also in the food industry. In the field of environmental monitoring, they control the quality of drinking water and the soil, thereby ensuring the preservation of environmental balance37.
Biosensors play a crucial role in developing treatment-focused approaches with their ability to detect and identify microorganisms such as bacteria, pathogens, and viruses. Through a mechanism that allows the detection and monitoring of chemical components without the need for blood tests, it becomes possible to track and control chemicals in the human body at any desired time38. When designed within a sensitive and efficient method, the detection of pathogens becomes more effective and precise, and determining infection and virulence doses holds significant importance in tracking diseases38,39.
Identification and quantitative assessment of pathogens are carried out by using immunological or DNA-based methods39. For pathogen detection, antibody- or antigen-based immunosensors, genosensors that use DNA samples, or aptasensors that use DNA or RNA aptamers are utilized40.
The fundamental and efficient functionality of biosensors comes from their ability to generate information about cell physiology by detecting tumor presence13. In this context, the mechanisms facilitating interactions with high-affinity biomolecules provide extensive sensitive screening and identification functions41. It is feasible to identify cancer in its early stages by measuring protein expression and secretion levels in the monitoring of tumor cells41. Lately, biosensors are being developed in autonomous and automatic forms to be put into practice in school offices, transportation centers, and similar social areas. They will be crucial in maintaining public health and safety as well as providing alerts in the event of future biological threats 42. The diversity of application areas for biosensors and the advancement of corresponding software technologies are increasing day by day42.
In conclusion, the preference for materials with faster, cost-effective, and stable mechanisms compared to traditional methods has become increasingly common. Biosensors with these capabilities function in a wide range of application areas. Their implementation methods, method diversity, and ability to deliver effective results in a short period are among their benefits. Today, with personalized design models, there is an increase in the possibility of efficient execution of individual health checks. Future benefits can be obtained by the diversification of application areas of biosensors, and adapting them to today’s technology can potentially generate positive societal impact.
References:
- Kawamura, A., & Miyata, T. (2016). Biosensors. In Biomaterials Nanoarchitectonics (pp. 157–176). Elsevier. https://doi.org/10.1016/B978-0-323-37127-8.00010-8
- Pacheco, J. G., Barroso, M. F., Nouws, H. P. A., Morais, S., & Delerue-Matos, C. (2017). Biosensors. In Current Developments in Biotechnology and Bioengineering (pp. 627–648). Elsevier. https://doi.org/10.1016/B978-0-444-63663-8.00021-5
- Leidner, L. (2020). Aiguo Wu, Waheed S. Khan (Eds.): Nanobiosensors: from design to applications. Analytical and Bioanalytical Chemistry, 412(30), 8239–8240. https://doi.org/10.1007/s00216-020-02975-0
- Wu, J., & Wu, Q. (2021). The Review of Biosensor and its Application in the Diagnosis of COVID-19. E3S Web of Conferences, 290, 03028. https://doi.org/10.1051/e3sconf/202129003028
- Malhotra, B. D., & Ali, Md. A. (2018). Nanomaterials in Biosensors. In Nanomaterials for Biosensors (pp. 1–74). Elsevier. https://doi.org/10.1016/B978-0-323-44923-6.00001-7
- Qazi, S., & Raza, K. (2020). Smart biosensors for an efficient point of care (PoC) health management. In Smart Biosensors in Medical Care (pp. 65–85). Elsevier. https://doi.org/10.1016/B978-0-12-820781-9.00004-8
- Martinkova, P., Kostelnik, A., Valek, T., & Pohanka, M. (2017). >Main streams in the Construction of Biosensors and Their Applications. International Journal of Electrochemical Science, 12(8), 7386–7403. https://doi.org/10.20964/2017.08.02
- Prajapati, S., Padhan, B., Amulyasai, B., & Sarkar, A. (2020). Nanotechnology-based sensors. In Biopolymer-Based Formulations (pp. 237–262). Elsevier. https://doi.org/10.1016/B978-0-12-816897-4.00011-4
- Sharma, A., Agrawal, A., Kumar, S., Awasthi, K. K., Awasthi, K., & Awasthi, A. (2021). Zinc oxide nanostructures–based biosensors. In Nanostructured Zinc Oxide (pp. 655–695). Elsevier. https://doi.org/10.1016/B978-0-12-818900-9.00002-4
- Song, M., Lin, X., Peng, Z., Xu, S., Jin, L., Zheng, X., & Luo, H. (2021). Materials and Methods of Biosensor Interfaces With Stability. Frontiers in Materials, 7. https://doi.org/10.3389/fmats.2020.583739
- Hammond, J. L., Formisano, N., Estrela, P., Carrara, S., & Tkac, J. (2016). Electrochemical biosensors and nanobiosensors. Essays in Biochemistry, 60(1), 69–80. https://doi.org/10.1042/EBC20150008
- Asal, M., Özen, Ö., Şahinler, M., & Polatoğlu, İ. (2018). Recent Developments in Enzyme, DNA and Immuno-Based Biosensors. Sensors, 18(6), 1924. https://doi.org/10.3390/s18061924
- Tetyana, P., Morgan Shumbula, P., & Njengele-Tetyana, Z. (2021). Biosensors: Design, Development and Applications. In Nanopores. IntechOpen. https://doi.org/10.5772/intechopen.97576
- Pan, H. M., Gonuguntla, S., Li, S., & Trau, D. (2017). 3.33 Conjugated Polymers for Biosensor Devices ☆. In Comprehensive Biomaterials II (pp. 716–754). Elsevier. https://doi.org/10.1016/B978-0-12-803581-8.10144-4
- Karimi-Maleh, H., Orooji, Y., Karimi, F., Alizadeh, M., Baghayeri, M., Rouhi, J., Tajik, S., Beitollahi, H., Agarwal, S., Gupta, V. K., Rajendran, S., Ayati, A., Fu, L., Sanati, A. L., Tanhaei, B., Sen, F., shabani-nooshabadi, M., Asrami, P. N., & Al-Othman, A. (2021). A critical review on the use of potentiometric based biosensors for biomarkers detection. Biosensors and Bioelectronics, 184, 113252. https://doi.org/10.1016/j.bios.2021.113252
- Chai, C., & Oh, S.-W. (2020). Electrochemical impedimetric biosensors for food safety. Food Science and Biotechnology, 29(7), 879–887. https://doi.org/10.1007/s10068-020-00776-w
- Chen, C., & Wang, J. (2020). Optical biosensors: an exhaustive and comprehensive review. The Analyst, 145(5), 1605–1628. https://doi.org/10.1039/C9AN01998G
- Garzón, V., Pinacho, D., Bustos, R.-H., Garzón, G., & Bustamante, S. (2019). Optical Biosensors for Therapeutic Drug Monitoring. Biosensors, 9(4), 132. https://doi.org/10.3390/bios9040132
- Pohanka, M. (2018). Overview of Piezoelectric Biosensors, Immunosensors and DNA Sensors and Their Applications. Materials, 11(3), 448. https://doi.org/10.3390/ma11030448
- Zu, H., Wu, H., & Wang, Q.-M. (2016). High-Temperature Piezoelectric Crystals for Acoustic Wave Sensor Applications. IEEE Transactions on Ultrasonics, Ferroelectrics, and Frequency Control, 63(3), 486–505. https://doi.org/10.1109/TUFFC.2016.2527599
- Sehgal, N., Mani, R. J., Dogra, N., & Katare, D. P. (2022). Biosensor-based early diagnosis of hepatic cancer. In Biosensor Based Advanced Cancer Diagnostics (pp. 97–111). Elsevier. https://doi.org/10.1016/B978-0-12-823424-2.00009-0
- Fu, Z., Lu, Y.-C., & Lai, J. J. (2019). Recent Advances in Biosensors for Nucleic Acid and Exosome Detection. Chonnam Medical Journal, 55(2), 86. https://doi.org/10.4068/cmj.2019.55.2.86
- Carvajal Barbosa, L., Insuasty Cepeda, D., León Torres, A. F., Arias Cortes, M. M., Rivera Monroy, Z. J., & Garcia Castaneda, J. E. (2021). Nucleic acid-based biosensors: analytical devices for prevention, diagnosis and treatment of diseases. Vitae, 28(3). https://doi.org/10.17533/udea.vitae.v28n3a347259
- Hua, Y., Ma, J., Li, D., & Wang, R. (2022). DNA-Based Biosensors for the Biochemical Analysis: A Review. Biosensors, 12(3), 183. https://doi.org/10.3390/bios12030183
- Shen, L., Wang, P., & Ke, Y. (2021). DNA Nanotechnology‐Based Biosensors and Therapeutics. Advanced Healthcare Materials, 10(15), 2002205. https://doi.org/10.1002/adhm.202002205
- Islam, M. N., Masud, M. K., Haque, M. H., Hossain, M. S. Al, Yamauchi, Y., Nguyen, N.-T., & Shiddiky, M. J. A. (2017). RNA Biomarkers: Diagnostic and Prognostic Potentials and Recent Developments of Electrochemical Biosensors. Small Methods, 1(7), 1700131. https://doi.org/10.1002/smtd.201700131
- Perumal, V., & Hashim, U. (2014). Advances in biosensors: Principle, architecture and applications. Journal of Applied Biomedicine, 12(1), 1–15. https://doi.org/10.1016/j.jab.2013.02.001
- Ning, Y., Hu, J., & Lu, F. (2020). Aptamers used for biosensors and targeted therapy. Biomedicine & Pharmacotherapy, 132, 110902. https://doi.org/10.1016/j.biopha.2020.110902
- Huang, X., Zhong, J., Ren, J., Wen, D., Zhao, W., & Huan, Y. (2019). A DNA aptamer recognizing MMP14 for in vivo and in vitro imaging identified by cell-SELEX. Oncology Letters. https://doi.org/10.3892/ol.2019.10282
- McConnell, E. M., Nguyen, J., & Li, Y. (2020). Aptamer-Based Biosensors for Environmental Monitoring. Frontiers in Chemistry, 8. https://doi.org/10.3389/fchem.2020.00434
- Aydin, M., Aydin, E. B., & Sezgintürk, M. K. (2021). Advances in immunosensor technology (pp. 1–62). https://doi.org/10.1016/bs.acc.2020.08.001
- Lim, S. A., & Ahmed, M. U. (2019). Introduction to Immunosensors. In Immunosensors (pp. 1–20). The Royal Society of Chemistry. https://doi.org/10.1039/9781788016162-00001
- Cristea, C., Florea, A., Tertis, M., & Sandulescu, R. (2015). Immunosensors. In Biosensors – Micro and Nanoscale Applications. InTech. https://doi.org/10.5772/60524
- Samadi Pakchin, P., Ghanbari, H., Saber, R., & Omidi, Y. (2018). Electrochemical immunosensor based on chitosan-gold nanoparticle/carbon nanotube as a platform and lactate oxidase as a label for detection of CA125 oncomarker. Biosensors and Bioelectronics, 122, 68–74. https://doi.org/10.1016/j.bios.2018.09.016
- Nguyen, H. H., Lee, S. H., Lee, U. J., Fermin, C. D., & Kim, M. (2019). Immobilized Enzymes in Biosensor Applications. Materials, 12(1), 121. https://doi.org/10.3390/ma12010121
- Campaña, A., Florez, S., Noguera, M., Fuentes, O., Ruiz Puentes, P., Cruz, J., & Osma, J. (2019). Enzyme-Based Electrochemical Biosensors for Microfluidic Platforms to Detect Pharmaceutical Residues in Wastewater. Biosensors, 9(1), 41. https://doi.org/10.3390/bios9010041
- Singh, S., Kumar, V., Dhanjal, D. S., Datta, S., Prasad, R., & Singh, J. (2020). Biological Biosensors for Monitoring and Diagnosis (pp. 317–335). https://doi.org/10.1007/978-981-15-2817-0_14
- Richards, R., Greimel, E., Kliemann, D., Koerte, I. K., Schulte-Körne, G., Reuter, M., & Wachinger, C. (2020). Increased hippocampal shape asymmetry and volumetric ventricular asymmetry in autism spectrum disorder. NeuroImage: Clinical, 26, 102207. https://doi.org/10.1016/j.nicl.2020.102207
- Cesewski, E., & Johnson, B. N. (2020). Electrochemical biosensors for pathogen detection. Biosensors and Bioelectronics, 159, 112214. https://doi.org/10.1016/j.bios.2020.112214
- Vidic, J., & Manzano, M. (2021). Electrochemical biosensors for rapid pathogen detection. Current Opinion in Electrochemistry, 29, 100750. https://doi.org/10.1016/j.coelec.2021.100750
- Bhalla, N., Jolly, P., Formisano, N., & Estrela, P. (2016). Introduction to biosensors. Essays in Biochemistry, 60(1), 1–8. https://doi.org/10.1042/EBC20150001
- Altug, H., Oh, S.-H., Maier, S. A., & Homola, J. (2022). Advances and applications of nanophotonic biosensors. Nature Nanotechnology, 17(1), 5–16. https://doi.org/10.1038/s41565-021-01045-5