Ayça DöngelT. , Elif DuymazE.
Since Yuri Gagarin performed the first human spacewalk in19611, research in the fields of astrobiology2, gravity biology3, space biology4, and space medicine5 has discovered novel biological responses to the space environment. Such as the National Aeronautics and Space Administration (NASA), the Japan Aerospace Exploration Agency (JAXA), the European Space Agency (ESA), the Russian State Space Operations Agency (ROSCOSMOS), the China National Space Administration (CNSA) and the Canadian Space Agency (CSA)6. Space agencies aimed to use science for the benefit of humanity6. To contribute to advances in science and technology on Earth, space agencies conduct studies for the ongoing updating of space research. For example; technology developed through space missions has led to the creation of solar cell and satellite navigation systems7-9. NASA Spinoffs aims to improve worldwide life with piezoelectric gravimeter and remote sensing toolkit technologies in 2022 goals9. In a similar manner, space biology has enabled the development of new technologies with a great deal of scientific knowledge contributing to terrestrial medicine and biological sciences10,11. Scientists plan to establish an interdisciplinary field called aerospace bioprocess engineering in the future12.
The term “spaceflight” refers to travel over the Karman line, which is 100 kilometers above sea level. The Karman line is an altitude above the atmosphere where the aerodynamic control surfaces of conventional aircraft are not dense enough for them to be effective; beyond that, there is space6,13. In terms of human health, spaceflight is classified into three categories: sub-orbital14, low earth orbit (LEO)15 and recon class missions16. Space radiation and microgravity are specifically the two main health risks connected to spaceflight17. Space radiation consists mainly of high-energy protons produced from solar particle events (SPE), heavy ions found in galactic cosmic rays (GCR), and secondary particles from interaction with the spacecraft shield18-20. Flights within the LEO can take advantage of the shielding effects of the Earth’s magnetosphere; however, beyond LEO, on upcoming exploration missions to the Moon, Mars, and other planets, crews are predicted to be exposed to increased GCR and possibly high-intensity SPE21,22. This review aims to outline the basic biological characteristics associated with their flight and the risks to human health that may be caused by space factors.
1. Space Factors
In order to establish functional bases on distant planets and missions beyond LEO, it is necessary to first identify the factors that make up the space environment and comprehensively determine their effects on living biology23. According to NASA, there are five main hazards to human spaceflight: radiation, microgravity, distance, closed or hostile ecosystems, and isolation/containment24.
1.1. Space Radiation
Ionizing radiation (IR) refers to short wavelengths (X-rays, gamma rays, cosmic rays, etc.) that cause significant adverse effects in the organism in a dose-dependent manner25. Space radiobiology (SPRB) is a specialized subfield that examines the physical characteristics of IRradiated from space27, in contrast to radiobiology, which is a multidisciplinary study of the biological impacts of IR26. Additionally, space missions often result in low-dose IR exposure; therefore, SPRB also examines some low-dose radiobiological events and how they affect the dangers related to radiation exposure27. One of the goals for SPRB is to simulate the space radiation environment on the surface of the Earth21,28.
1.2. Microgravity
It is believed that the physical constant of gravity (g=9.1 m/s2) has not changed in the past four billion years29. The term microgravity also referred to as weightlessness, is commonly used to describe accelerations close to 10–3-10–6g30,31. Microgravity is one of the major factors contributing to the harsh space environment, which causes several significant changes in living systems. First of all, during the first few days in orbit, 60–80% of astronauts experience space adaptation syndrome (SAS), commonly known as space sickness (SMS)5. Research opportunities exist that will help us better understand the biological impacts of this factor on cells in vitro by recreating microgravity with Earth-based devices32-34.
1.3. Distance
The phenomenon of “disappearing earth” is expressed as an increase in longing and melancholy depending on the distance from the Earth35. As moving away from Earth, work is being carried out to address technical problems such as the difficulty of real-time communication with a delay of up to 20 minutes and limitations in health services36. Long-distance space exploration missions (LDSEMs) in particular attempt to maintain crew members’ physical and psychological health in order to standardize crew dynamics37.
1.4. Closed or Hostile Ecosystems
The ecosystem inside the spacecraft can affect astronaut health if necessary precautions are not taken24. Stress hormone levels can increase in a closed environment. It has also been reported that astronauts’ immune systems and metabolisms are affected by space flights in a closed ecosystem24,38. In particular, the ventilation criteria of the spacecraft environment are critical to avoid exposure to various pollutants and celestial space dust39,40.
1.5. Isolation/Imprisonment
In conditions of imprisonment and isolation, human psychology differs considerably. In long-duration space exploration missions, it has been reported that behavioral health risks need to be addressed for mission success. Earth-based studies simulating isolation in space in analog environments have shown that social isolation can lead to neurological disorders, an increase in anxiety, and immune system disorders41-44.
2. Human Health in Space
Six features of spaceflight biology have been identified from studies on model organisms and astronaut health that provide insight into the fundamental molecular changes that occur during space travel. Oxidative stress, DNA damage, mitochondrial dysregulation, epigenetic alterations, telomere length changes, and microbiome adjustments are some of these features (Figure 1)24.
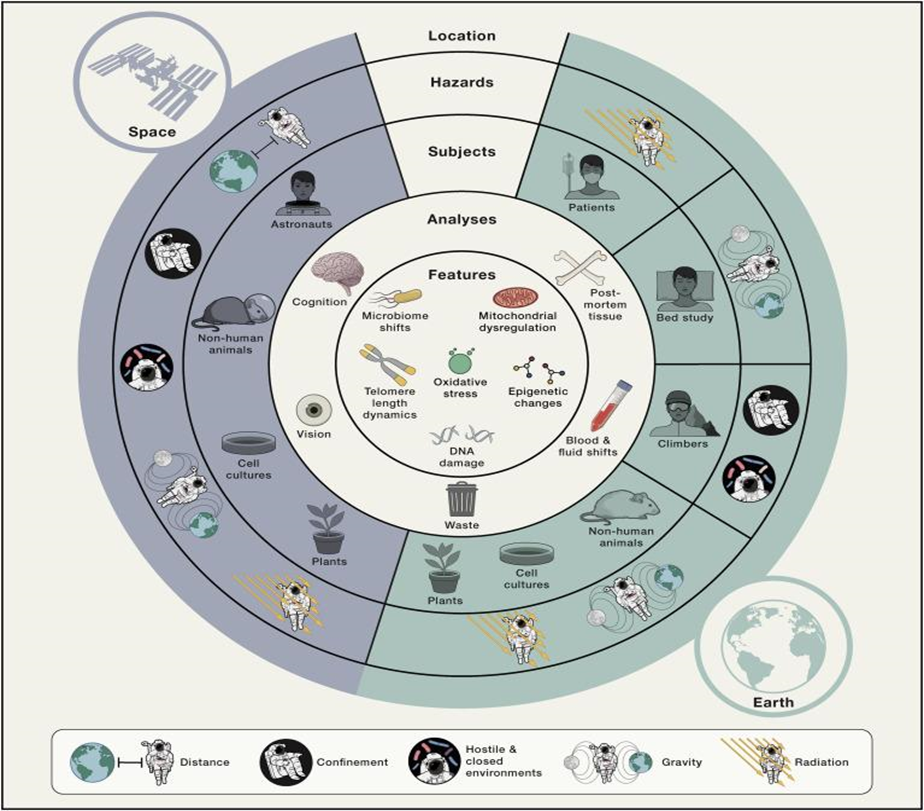
Figure 1. Space factors and their biological effects on living things24. The physiological/biological effects of the dangers created by the space environment for living things and their relations with each other have been mapped.
2.1. Skeletal Muscle system
Research conducted before, during, and after spaceflight has demonstrated that exposure to microgravity disrupts various physiological systems, with the skeletal and muscular systems being particularly affected45,48. Decreases in mechanical loading on the skeleton due to microgravity cause deterioration of the skeletal architecture leading to significant losses in bone mineral density (BMD) and its bone strength. Biochemical studies have shown that bone metabolism changes during spaceflight. Biomarkers of bone resorption increase, while biomarkers of bone formation decrease, resulting in net bone loss. In addition to microgravity, it is claimed that lack of exercise, low nutritional diversity, and mobility in limited space in spacecraft can contribute to bone 47-52. Muscular atrophy is another health issue caused by microgravity. It is stated that the decrease in muscle volume due to continuous exposure to microgravity during long-term space flight may increase the risk of astronaut injury, especially in the spine and lower extremities, while on duty, after returning to earth, or later in life53,54.
2.2. Cardiovascular System
Beyond LEO, the cardiovascular system (CVS) is expected to have deleterious effects from exposure to space radiation, including cardiac remodeling, fibrosis, and (micro)vascular damage55. Microgravity has various effects on CVS physiology, such as reduced circulating blood volumes, decreased arterial blood diastolic pressure, reduced left ventricular size, and post-flight orthostatic intolerance56-58. Blood and other body fluids go from the lower extremities to the upper body during spaceflight because there is no hydrostatic gradient under microgravity circumstances. Positional changes in body fluids cause physiological changes that are evident as a puffy face linked with facial edema chicken legs due to diminished leg volume59. In addition, changes occurring in the space environment CVS can promote oxidative stress and inflammation, which can disturb vascular endothelial cells (ECs) and accelerate the progression of cardiovascular disease60.
2.3. Nervous System
Long-term exploration of space places significant importance on maintaining the health of the brain and central nervous system (CNS), as sensorimotor and cognitive functions are essential for many important tasks61. The human brain experiences a variety of changes in the space environment according to research on astronauts returning from missions to the International Space Station (ISS). Such as micro and macro structural changes in gray and white matter, changes in cerebrospinal fluid (CSF) and fluid distribution, and changes in behavioral and cognitive performance62-64. For instance, exposure of the vestibular/sensorimotor system to real or simulated microgravity caused structural and functional changes such as conflicts between actual and expected signals collected from the external environment65-67. Acute consequences of space radiation exposure during missions beyond LEO include a broad spectrum and can include everything from impacted cognitive function to CNS damage and even death if astronauts are exposed to extremely high doses of particles. The long-term effects of radiation exposure may include an increased risk of cancer development or permanent damage to brain structures62,68. Long-term spaceflight increases the brain’s total intracranial volume; it has also been discovered to cause structural changes in the brain, as well as conditions like spaceflight-associated neuro-ocular syndrome (SANS), formerly known as visual impairment and intracranial pressure (VIIP), cognitive impairments, or performance-related effects like neuro vestibular issues69. The long-term isolation environment in space missions also has psychological and physiological effects. Isolation studies such as the Mars500 have shed light on microstructural changes in the brain’s white matter63,70. Misalignment of the circadian clock system, which is among the physiological functions affected by spaceflight, has been reported to affect astronauts’ health, sleep/wake cycles, and performance24.
2.4. Other Systems
The primary respiratory organ, the lungs, carries out gas exchange through passive diffusion, which is measured by the ventilation-perfusion ratio (V/Q), which calls for the proper mixing of inhaled gas and blood flow across the alveolar membrane. In the normal human lung, gravity couples ventilation with perfusion through mechanical effects; therefore, it is stated that microgravity may cause organ deformation by increasing the lung weight due to fluid accumulation. In addition, it has been pointed out that exposure to dust particles in the aerospace environment can cause particulate accumulation in the respiratory tract71. Long-term reports have connected astronauts’ opportunistic infections to immune system disorders, another important regulator impacted by the space environment72. It is anticipated that crew members on exploration-class space missions will experience clinical risk due to persistent reductions in the function of particular innate or adaptive immune cells or changes in cytokine production profiles. It is also believed that the space environment increases the risk of cancer due to genetic changes73-75. Reproductive systems and fertility are on the agenda of scientists in terms of future colonies on other planets or deep space travels, but microgravity and radiation are on the reproductive system. It has only been shown in animal models that it may have negative effects on its functions76,77. A recent study reported that normal reproductive function occurs in mouse sperm frozen under space radiation conditions78. The gut microbiome, which is found in the digestive tract, is crucial for preserving astronaut health while in space, just as it is for people on Earth. Studies involving both animal and human participants have been carried out to track alterations in the gut microbiota under actual or simulated spaceflight circumstances. Microbiome dysbiosis, gastrointestinal problems, and infection are all connected to a variety of clinical outcomes, including neurological and metabolic conditions38,79. Other illnesses that may be dangerous to human health in space are documented to include hormonal dysfunction83, skin issues81, lipid dysregulation24, liver damage, and renal nephrolithiasis (kidney stones)80.
Throughout human history, there has been great interest in and investigation into phenomena beyond the atmosphere. Significant breakthroughs have been made in the exploration of other planets and deep space, from the first man walking on the Moon to the present day. These breakthroughs have brought along technological developments in various branches of science, including biological sciences around the world. Today, deep space travels planned, especially to Mars, bring along concerns about the adaptation of humans to the space environment. Long-term space missions and voyages face obstacles due to the hazards of the space environment and potential diseases. Scientists devote significant effort to creating systems that safeguard and sustain human health during deep space exploration.
References:
- Furukawa, S., Nagamatsu, A., Nenoi, M., Fujimori, A., Kakinuma, S., Katsube, T., Wang, B., Tsuruoka, C., Shirai, T., Nakamura, A. J., Sakaue-Sawano, A., Miyawaki, A., Harada, H., Kobayashi, M., Kobayashi, J., Kunieda, T., Funayama, T., Suzuki, M., Miyamoto, T., … Takahashi, A. (2020). Space Radiation Biology for “Living in Space.” BioMed Research International, 2020. https://doi.org/10.1155/2020/4703286
- Prasad, B., Richter, P., Vadakedath, N., Haag, F. W. M., Strauch, S. M., Mancinelli, R., Schwarzwälder, A., Etcheparre, E., Gaume, N., & Lebert, M. (2021). How the space environment influences organisms: an astrobiological perspective and review. International Journal of Astrobiology, 20(2), 159–177. https://doi.org/10.1017/S1473550421000057
- Grimm, D. (2019). Guest Edited Collection: Gravitational biology and space medicine. Scientific Reports 2019 9:1, 9(1), 1–4. https://doi.org/10.1038/s41598-019-51231-8
- Vandenbrink, J. P., & Kiss, J. Z. (2016). Space, the final frontier: A critical review of recent experiments performed in microgravity. Plant Science : An International Journal of Experimental Plant Biology, 243, 115. https://doi.org/10.1016/J.PLANTSCI.2015.11.004
- Hodkinson, P. D., Anderton, R. A., Posselt, B. N., & Fong, K. J. (2017). An overview of space medicine. British Journal of Anaesthesia, 119, i143–i153. https://doi.org/10.1093/BJA/AEX336
- Smith, M. G., Kelley, M., & Basner, M. (2020). A brief history of spaceflight from 1961 to 2020: An analysis of missions and astronaut demographics. Acta Astronautica, 175, 290. https://doi.org/10.1016/J.ACTAASTRO.2020.06.004
- Maiwald, V., Schubert, D., Quantius, D., & Zabel, P. (2021). From space back to Earth: supporting sustainable development with spaceflight technologies. Sustainable Earth 2021 4:1, 4(1), 1–16. https://doi.org/10.1186/S42055-021-00042-9
- Dietrich, D., Dekova, R., Davy, S., Fahrni, G., & Geissbühler, A. (2018). Applications of Space Technologies to Global Health: Scoping Review. Journal of Medical Internet Research, 20(6). https://doi.org/10.2196/JMIR.9458
- NASA Spinoff 2022 Feature Stennis Space Center Technologies | NASA. (n.d.-i). Retrieved July 15, 2022, from https://www.nasa.gov/centers/stennis/news/releases/2022/NASA-Spinoff-2022-Feature-Stennis-Space-Center-Technologies
- Orlov, O. I., Belakovskiy, M. S., Kussmaul, A. R., & Tomilovskaya, E. S. (2022). Using the Possibilities of Russian Space Medicine for Terrestrial Healthcare. Frontiers in Physiology, 0, 947. https://doi.org/10.3389/FPHYS.2022.921487
- Tran, Q. D., Tran, V., Toh, L. S., Williams, P. M., Tran, N. N., & Hessel, V. (2021). Space Medicines for Space Health. ACS Medicinal Chemistry Letters. https://doi.org/10.1021/acsmedchemlett.1c00681
- Berliner, A. J., Lipsky, I., Ho, D., Hilzinger, J. M., Vengerova, G., Makrygiorgos, G., McNulty, M. J., Yates, K., Averesch, N. J. H., Cockell, C. S., Wallentine, T., Seefeldt, L. C., Criddle, C. S., Nandi, S., McDonald, K. A., Menezes, A. A., Mesbah, A., & Arkin, A. P. (2022). Space bioprocess engineering on the horizon. Communications Engineering 2022 1:1, 1(1), 1–8. https://doi.org/10.1038/s44172-022-00012-9
- BARRATT, M. R. (2020). THE BERT & PEGGY DUPONT LECTURE: THE HUMAN IN SPACE: A NEW PHYSIOLOGY. Transactions of the American Clinical and Climatological Association, 131, 201. https://www.ncbi.nlm.nih.gov/pmc/articles/PMC7358469/
- Pollock, R. D., Jolley, C. J., Abid, N., Couper, J. H., Estrada-Petrocelli, L., Hodkinson, P. D., Leonhardt, S., Magor-Elliott, S., Menden, T., Rafferty, G., Richmond, G., Robbins, P. A., Ritchie, G. A. D., Segal, M. J., Stevenson, A. T., Tank, H. D., & Smith, T. G. (2021). Pulmonary Effects of Sustained Periods of High-G Acceleration Relevant to Suborbital Spaceflight. Aerospace Medicine and Human Performance, 92(8), 633–641. https://doi.org/10.3357/AMHP.5790.2021
- Paul, A. M., Cheng-Campbell, M., Blaber, E. A., Anand, S., Bhattacharya, S., Zwart, S. R., Crucian, B. E., Smith, S. M., Meller, R., Grabham, P., & Beheshti, A. (2020). Beyond Low-Earth Orbit: Characterizing Immune and microRNA Differentials following Simulated Deep Spaceflight Conditions in Mice. IScience, 23(12). https://doi.org/10.1016/J.ISCI.2020.101747
- Crucian, B. E., Choukèr, A., Simpson, R. J., Mehta, S., Marshall, G., Smith, S. M., Zwart, S. R., Heer, M., Ponomarev, S., Whitmire, A., Frippiat, J. P., Douglas, G., Lorenzi, H., Buchheim, J. I., Makedonas, G., Ginsburg, G. S., Mark Ott, C., Pierson, D. L., Krieger, S. S., … Sams, C. (2018). Immune system dysregulation during spaceflight: Potential countermeasures for deep space exploration missions. Frontiers in Immunology, 9(JUN), 1437. https://doi.org/10.3389/fimmu.2018.01437
- Patel, S. (2020). The effects of microgravity and space radiation on cardiovascular health: From low-Earth orbit and beyond. International Journal of Cardiology. Heart & Vasculature, 30, 100595. https://doi.org/10.1016/J.IJCHA.2020.100595
- Carnell, L. S. (2020). Spaceflight medical countermeasures: a strategic approach for mitigating effects from solar particle events. Https://Doi.Org/10.1080/09553002.2020.1820603, 97(S1), S125–S131. https://doi.org/10.1080/09553002.2020.1820603
- Slaba, T. C., & Singleterry, R. C. (2020). Correct modeling results are needed to inform mission planning and shield design. Life Sciences in Space Research, 25, 143–147. https://doi.org/10.1016/J.LSSR.2019.11.001
- Loffredo, F., Vardaci, E., Bianco, D., Di Nitto, A., & Quarto, M. (2022). Protons Interaction with Nomex Target: Secondary Radiation from a Monte Carlo Simulation with Geant4. Applied Sciences 2022, Vol. 12, Page 2643, 12(5), 2643. https://doi.org/10.3390/APP12052643
- Simonsen, L. C., Slaba, T. C., Guida, P., & Rusek, A. (2020). NASA’s first ground-based Galactic Cosmic Ray Simulator: Enabling a new era in space radiobiology research. PLoS Biology, 18(5). https://doi.org/10.1371/JOURNAL.PBIO.3000669
- Norbury, J. W., Slaba, T. C., Aghara, S., Badavi, F. F., Blattnig, S. R., Clowdsley, M. S., Heilbronn, L. H., Lee, K., Maung, K. M., Mertens, C. J., Miller, J., Norman, R. B., Sandridge, C. A., Singleterry, R., Sobolevsky, N., Spangler, J. L., Townsend, L. W., Werneth, C. M., Whitman, K., … Zeitlin, C. (2019). Advances in space radiation physics and transport at NASA. Life Sciences in Space Research, 22, 98–124. https://doi.org/10.1016/J.LSSR.2019.07.003
- Kanapskyte, A., Hawkins, E. M., Liddell, L. C., Bhardwaj, S. R., Gentry, D., & Santa Maria, S. R. (2021). Space Biology Research and Biosensor Technologies: Past, Present, and Future. Biosensors, 11(2). https://doi.org/10.3390/BIOS11020038
- Afshinnekoo, E., Scott, R. T., MacKay, M. J., Pariset, E., Cekanaviciute, E., Barker, R., Gilroy, S., Hassane, D., Smith, S. M., Zwart, S. R., Nelman-Gonzalez, M., Crucian, B. E., Ponomarev, S. A., Orlov, O. I., Shiba, D., Muratani, M., Yamamoto, M., Richards, S. E., Vaishampayan, P. A., … Beheshti, A. (2020). Fundamental Biological Features of Spaceflight: Advancing the Field to Enable Deep Space Exploration. Cell, 183(5), 1162. https://doi.org/10.1016/J.CELL.2020.10.050
- Tuieng, R. J., Cartmell, S. H., Kirwan, C. C., & Sherratt, M. J. (2021). The Effects of Ionising and Non-Ionising Electromagnetic Radiation on Extracellular Matrix Proteins. Cells, 10(11). https://doi.org/10.3390/CELLS10113041
- Kirsch, D. G., Diehn, M., Kesarwala, A. H., Maity, A., Morgan, M. A., Schwarz, J. K., Bristow, R., Demaria, S., Eke, I., Griffin, R. J., Haas-Kogan, D., Higgins, G. S., Kimmelman, A. C., Kimple, R. J., Lombaert, I. M., Ma, L., Marples, B., Pajonk, F., Park, C. C., … Bernhard, E. J. (2018). The Future of Radiobiology. JNCI Journal of the National Cancer Institute, 110(4), 329. https://doi.org/10.1093/JNCI/DJX231
- Strigari, L., Strolin, S., Morganti, A. G., & Bartoloni, A. (2021). Dose-Effects Models for Space Radiobiology: An Overview on Dose-Effect Relationships. Frontiers in Public Health, 9, 733337. https://doi.org/10.3389/FPUBH.2021.733337
- Walsh, L., Schneider, U., Fogtman, A., Kausch, C., McKenna-Lawlor, S., Narici, L., Ngo-Anh, J., Reitz, G., Sabatier, L., Santin, G., Sihver, L., Straube, U., Weber, U., & Durante, M. (2019). Research plans in Europe for radiation health hazard assessment in exploratory space missions. Life Sciences in Space Research, 21, 73–82. https://doi.org/10.1016/J.LSSR.2019.04.002
- Shapshak, P. (2018). Astrobiology – an opposing view. Bioinformation, 14(6), 346–349. https://doi.org/10.6026/97320630014346
- Huang, B., Li, D. G., Huang, Y., & Liu, C. T. (2018). Effects of spaceflight and simulated microgravity on microbial growth and secondary metabolism. Military Medical Research, 5(1). https://doi.org/10.1186/S40779-018-0162-9
- Demontis, G. C., Germani, M. M., Caiani, E. G., Barravecchia, I., Passino, C., & Angeloni, D. (2017). Human Pathophysiological Adaptations to the Space Environment. Frontiers in Physiology, 8(AUG), 547. https://doi.org/10.3389/FPHYS.2017.00547
- Garrett-Bakelman, F. E., Darshi, M., Green, S. J., Gur, R. C., Lin, L., Macias, B. R., McKenna, M. J., Meydan, C., Mishra, T., Nasrini, J., Piening, B. D., Rizzardi, L. F., Sharma, K., Siamwala, J. H., Taylor, L., Vitaterna, M. H., Afkarian, M., Afshinnekoo, E., Ahadi, S., … Turek, F. W. (2019). The NASA twins study: A multidimensional analysis of a year-long human spaceflight. Science, 364(6436). https://doi.org/10.1126/science.aau8650
- Anil-Inevi, M., Sarigil, O., Kizilkaya, M., Mese, G., Tekin, H. C., & Ozcivici, E. (2020). Stem Cell Culture Under Simulated Microgravity. Advances in Experimental Medicine and Biology, 1298, 105–132. https://doi.org/10.1007/5584_2020_539
- Yatagai, F., Honma, M., Dohmae, N., & Ishioka, N. (2019). Biological effects of space environmental factors: A possible interaction between space radiation and microgravity. Life Sciences in Space Research, 20, 113–123. https://doi.org/10.1016/J.LSSR.2018.10.004
- Gushin, V., Ryumin, O., Karpova, O., Rozanov, I., Shved, D., & Yusupova, A. (2021). Prospects for Psychological Support in Interplanetary Expeditions. Frontiers in Physiology, 12. https://doi.org/10.3389/FPHYS.2021.750414
- Doarn, C., Polk, J., & Shepanek, M. (2019). Health challenges including behavioral problems in long-duration spaceflight. Neurology India, 67(Supplement), S190–S195. https://doi.org/10.4103/0028-3886.259116
- Bell, S. T., Brown, S. G., & Mitchell, T. (2019). What we know about team dynamics for long-distance space missions: A systematic review of analog research. Frontiers in Psychology, 10(MAY). https://doi.org/10.3389/FPSYG.2019.00811/
- Turroni, S., Magnani, M., KC, P., Lesnik, P., Vidal, H., & Heer, M. (2020). Gut Microbiome and Space Travelers’ Health: State of the Art and Possible Pro/Prebiotic Strategies for Long-Term Space Missions. Frontiers in Physiology, 11. https://doi.org/10.3389/FPHYS.2020.553929
- Checinska Sielaff, A., Urbaniak, C., Mohan, G. B. M., Stepanov, V. G., Tran, Q., Wood, J. M., Minich, J., McDonald, D., Mayer, T., Knight, R., Karouia, F., Fox, G. E., & Venkateswaran, K. (2019). Characterization of the total and viable bacterial and fungal communities associated with the International Space Station surfaces. Microbiome, 7(1). https://doi.org/10.1186/S40168-019-0666-X
- Kobrick, R. L., & Agui, J. H. (2019). Preparing for planetary surface exploration by measuring habitat dust intrusion with filter tests during an analogue Mars mission. Acta Astronautica, 160, 297–309. https://doi.org/10.1016/J.ACTAASTRO.2019.04.040
- Zivi, P., De Gennaro, L., & Ferlazzo, F. (2020). Sleep in Isolated, Confined, and Extreme (ICE): A Review on the Different Factors Affecting Human Sleep in ICE. Frontiers in Neuroscience, 14, 851. https://doi.org/10.3389/fnins.2020.00851
- Mhatre, S. D., Iyer, J., Puukila, S., Paul, A. M., Tahimic, C. G. T., Rubinstein, L., Lowe, M., Alwood, J. S., Sowa, M. B., Bhattacharya, S., Globus, R. K., & Ronca, A. E. (2022). Neuro-consequences of the spaceflight environment. Neuroscience & Biobehavioral Reviews, 132, 908–935. https://doi.org/10.1016/J.NEUBIOREV.2021.09.055
- Patel, Z. S., Brunstetter, T. J., Tarver, W. J., Whitmire, A. M., Zwart, S. R., Smith, S. M., & Huff, J. L. (2020). Red risks for a journey to the red planet: The highest priority human health risks for a mission to Mars. Npj Microgravity 2020 6:1, 6(1), 1–13. https://doi.org/10.1038/s41526-020-00124-6
- Suedfeld, P. (2018). Antarctica and space as psychosocial analogues. REACH, 9–12, 1–4. https://doi.org/10.1016/J.REACH.2018.11.001
- Lee, S. J., Lehar, A., Meir, J. U., Koch, C., Morgan, A., Warren, L. E., Rydzik, R., Youngstrom, D. W., Chandok, H., George, J., Gogain, J., Michaud, M., Stoklasek, T. A., Liu, Y., & Germain-Lee, E. L. (2020). Targeting myostatin/activin A protects against skeletal muscle and bone loss during spaceflight. Proceedings of the National Academy of Sciences of the United States of America, 117(38), 23942–23951. https://doi.org/10.1073/pnas.2014716117
- Rittweger, J., Albracht, K., Flück, M., Ruoss, S., Brocca, L., Longa, E., Moriggi, M., Seynnes, O., Di Giulio, I., Tenori, L., Vignoli, A., Capri, M., Gelfi, C., Luchinat, C., Francheschi, C., Bottinelli, R., Cerretelli, P., & Narici, M. (2018). Sarcolab pilot study into skeletal muscle’s adaptation to long-term spaceflight. NPJ Microgravity, 4(1), 18. https://doi.org/10.1038/S41526-018-0052-1
- Stavnichuk, M., Mikolajewicz, N., Corlett, T., Morris, M., & Komarova, S. V. (2020). A systematic review and meta-analysis of bone loss in space travelers. Npj Microgravity 2020 6:1, 6(1), 1–9. https://doi.org/10.1038/s41526-020-0103-2
- Vico, L., & Hargens, A. (2018). Skeletal changes during and after spaceflight. Nature Reviews Rheumatology 2018 14:4, 14(4), 229–245. https://doi.org/10.1038/nrrheum.2018.37
- Vico, L., van Rietbergen, B., Vilayphiou, N., Linossier, M. T., Locrelle, H., Normand, M., Zouch, M., Gerbaix, M., Bonnet, N., Novikov, V., Thomas, T., & Vassilieva, G. (2017). Cortical and Trabecular Bone Microstructure Did Not Recover at Weight-Bearing Skeletal Sites and Progressively Deteriorated at Non-Weight-Bearing Sites During the Year Following International Space Station Missions. Journal of Bone and Mineral Research, 32(10), 2010–2021. https://doi.org/10.1002/JBMR.3188
- Gabel, L., Liphardt, A. M., Hulme, P. A., Heer, M., Zwart, S. R., Sibonga, J. D., Smith, S. M., & Boyd, S. K. (2022). Pre-flight exercise and bone metabolism predict unloading-induced bone loss due to spaceflight. British Journal of Sports Medicine, 56(4), 196–203. https://doi.org/10.1136/BJSPORTS-2020-103602
- Lang, T., Van Loon, J. J. W. A., Bloomfield, S., Vico, L., Chopard, A., Rittweger, J., Kyparos, A., Blottner, D., Vuori, I., Gerzer, R., & Cavanagh, P. R. (2017). Towards human exploration of space: the THESEUS review series on muscle and bone research priorities. NPJ Microgravity, 3(1). https://doi.org/10.1038/S41526-017-0013-0
- Genah, S., Monici, M., & Morbidelli, L. (2021). The Effect of Space Travel on Bone Metabolism: Considerations on Today’s Major Challenges and Advances in Pharmacology. International Journal of Molecular Sciences, 22(9). https://doi.org/10.3390/IJMS22094585
- Comfort, P., McMahon, J. J., Jones, P. A., Cuthbert, M., Kendall, K., Lake, J. P., & Haff, G. G. (2021). Effects of Spaceflight on Musculoskeletal Health: A Systematic Review and Meta-analysis, Considerations for Interplanetary Travel. Sports Medicine, 51(10), 2097–2114. https://doi.org/10.1007/s40279-021-01496-9
- Furukawa, S., Chatani, M., Higashitani, A., Higashibata, A., Kawano, F., Nikawa, T., Numaga-Tomita, T., Ogura, T., Sato, F., Sehara-Fujisawa, A., Shinohara, M., Shimazu, T., Takahashi, S., & Watanabe-Takano, H. (2021). Findings from recent studies by the Japan Aerospace Exploration Agency examining musculoskeletal atrophy in space and on Earth. Npj Microgravity 2021 7:1, 7(1), 1–10. https://doi.org/10.1038/s41526-021-00145-9
- Vernice, N. A., Meydan, C., Afshinnekoo, E., & Mason, C. E. (2020). Long-term spaceflight and the cardiovascular system. Precision Clinical Medicine, 3(4), 284–291. https://doi.org/10.1093/PCMEDI/PBAA022
- MacNamara, J. P., Dias, K. A., Sarma, S., Lee, S. M. C., Martin, D., Romeijn, M., Zaha, V. G., & Levine, B. D. (2021). Cardiac Effects of Repeated Weightlessness during Extreme Duration Swimming Compared with Spaceflight. Circulation, 143(15), 1533–1535. https://doi.org/10.1161/CIRCULATIONAHA.120.050418
- Rabineau, J., Hossein, A., Landreani, F., Haut, B., Mulder, E., Luchitskaya, E., Tank, J., Caiani, E. G., van de Borne, P., & Migeotte, P. F. (2020). Cardiovascular adaptation to simulated microgravity and countermeasure efficacy assessed by ballistocardiography and seismocardiography. Scientific Reports 2020 10:1, 10(1), 1–13. https://doi.org/10.1038/s41598-020-74150-5
- Iwase, S., Nishimura, N., Tanaka, K., & Mano, T. (2020). Effects of Microgravity on Human Physiology. Beyond LEO – Human Health Issues for Deep Space Exploration [Working Title]. https://doi.org/10.5772/INTECHOPEN.90700
- Burki, T. (2021). The final frontier: health in space. Lancet (London, England), 398(10296), 199–200. https://doi.org/10.1016/S0140-6736(21)01644-5
- Lee, S. M. C., Ribeiro, L. C., Martin, D. S., Zwart, S. R., Feiveson, A. H., Laurie, S. S., Macias, B. R., Crucian, B. E., Krieger, S., Weber, D., Grune, T., Platts, S. H., Smith, S. M., & Stenger, M. B. (2020). Arterial structure and function during and after long-duration spaceflight. Journal of Applied Physiology, 129(1), 108–123. https://doi.org/10.1152/japplphysiol.00550.2019
- Tays, G. D., Hupfeld, K. E., McGregor, H. R., Salazar, A. P., De Dios, Y. E., Beltran, N. E., Reuter-Lorenz, P. A., Kofman, I. S., Wood, S. J., Bloomberg, J. J., Mulavara, A. P., & Seidler, R. D. (2021). The Effects of Long Duration Spaceflight on Sensorimotor Control and Cognition. Frontiers in Neural Circuits, 15, 723504. https://doi.org/10.3389/fncir.2021.723504
- Jandial, R., Hoshide, R., Waters, J. D., & Limoli, C. L. (2018). Space–brain: The negative effects of space exposure on the central nervous system. Surgical Neurology International, 9(1). https://doi.org/10.4103/SNI.SNI_250_17
- Roy-O’Reilly, M., Mulavara, A., & Williams, T. (2021). A review of alterations to the brain during spaceflight and the potential relevance to crew in long-duration space exploration. NPJ Microgravity, 7(1). https://doi.org/10.1038/S41526-021-00133-Z
- Lee, J. K., Koppelmans, V., Pasternak, O., Beltran, N. E., Kofman, I. S., Dios, Y. E. De, Mulder, E. R., Mulavara, A. P., Bloomberg, J. J., & Seidler, R. D. (2021). Effects of Spaceflight Stressors on Brain Volume, Microstructure, and Intracranial Fluid Distribution. Cerebral Cortex Communications, 2(2), 1–14. https://doi.org/10.1093/TEXCOM/TGAB022
- Ganapathy, K., Da Rosa, M., & Russomano, T. (2019). Neurological changes in outer space. Neurology India, 67(1), 37. https://pubmed.ncbi.nlm.nih.gov/30860089/
- Russomano, T., Da Rosa, M., & Dos Santos, M. (2019). Space motion sickness: A common neurovestibular dysfunction in microgravity. Neurology India, 67(8), 214. https://doi.org/10.4103/0028-3886.259127
- Dixon, J. B., & Clark, T. K. (2020). Sensorimotor impairment from a new analog of spaceflight-altered neurovestibular cues. Journal of Neurophysiology, 123(1), 209–223. https://doi.org/10.1152/jn.00156.2019
- Davis, C. M., Allen, A. R., & Bowles, D. E. (2021). Consequences of space radiation on the brain and cardiovascular system. Journal of Environmental Science and Health. Part C, Toxicology and Carcinogenesis, 39(2), 180–218. https://doi.org/10.1080/26896583.2021.1891825
- Michael, A. P. (2018). Spaceflight Induced Changes in the Central Nervous System. Into Space – A Journey of How Humans Adapt and Live in Microgravity. https://doi.org/10.5772/INTECHOPEN.74232
- Brem, C., Lutz, J., Vollmar, C., Feuerecker, M., Strewe, C., Nichiporuk, I., Vassilieva, G., Schelling, G., & Choukér, A. (2020). Changes of brain DTI in healthy human subjects after 520 days isolation and confinement on a simulated mission to Mars. Life Sciences in Space Research, 24, 83–90. https://doi.org/10.1016/J.LSSR.2019.09.004
- Prisk, G. K. (2019). Pulmonary challenges of prolonged journeys to space: taking your lungs to the moon. The Medical Journal of Australia, 211(6), 271. https://doi.org/10.5694/MJA2.50312
- Mann, V., Sundaresan, A., Mehta, S., Crucian, B., Doursout, M., & Devakottai, S. (2019). Effects of microgravity and other space stressors in immunosuppression and viral reactivation with potential nervous system involvement. Neurology India, 67(8), 198. https://doi.org/10.4103/0028-3886.259125
- Akiyama, T., Horie, K., Hinoi, E., Hiraiwa, M., Kato, A., Maekawa, Y., Takahashi, A., & Furukawa, S. (2020). How does spaceflight affect the acquired immune system? NPJ Microgravity, 6(1). https://doi.org/10.1038/S41526-020-0104-1
- Sarkar, R., & Pampaloni, F. (2022). In Vitro Models of Bone Marrow Remodelling and Immune Dysfunction in Space: Present State and Future Directions. Biomedicines 2022, Vol. 10, Page 766, 10(4), 766. https://doi.org/10.3390/BIOMEDICINES10040766
- Cucinotta, F. A., & Saganti, P. B. (2022). Race and ethnic group dependent space radiation cancer risk predictions. Scientific Reports 2022 12:1, 12(1), 1–10. https://doi.org/10.1038/s41598-022-06105-x
- Mishra, B., & Luderer, U. (2019). Reproductive hazards of space travel in women and men. Nature Reviews. Endocrinology, 15(12), 713. https://doi.org/10.1038/S41574-019-0267-6
- Proshchina, A., Gulimova, V., Kharlamova, A., Krivova, Y., Besova, N., Berdiev, R., & Saveliev, S. (2021). Reproduction and the Early Development of Vertebrates in Space: Problems, Results, Opportunities. Life 2021, Vol. 11, Page 109, 11(2), 109. https://doi.org/10.3390/LIFE11020109
- Wakayama, S., Ito, D., Kamada, Y., Shimazu, T., Suzuki, T., Nagamatsu, A., Araki, R., Ishikawa, T., Kamimura, S., Hirose, N., Kazama, K., Yang, L., Inoue, R., Kikuchi, Y., Hayashi, E., Emura, R., Watanabe, R., Nagatomo, H., Suzuki, H., … Wakayama, T. (2021). Evaluating the long-term effect of space radiation on the reproductive normality of mammalian sperm preserved on the International Space Station. Science Advances, 7(24), 5554–5565. https://doi.org/10.1126/sciadv.abg5554
- Kirkpatrick, A. W., Hamilton, D. R., McKee, J. L., McDonald, B., Pelosi, P., Ball, C. G., Roberts, D. J., McBeth, P. B., Coccolini, F., Ansaloni, L., Peireira, B. M., Sugrue, M., Campbell, M. R., Kimball, E. J., & Malbrain, M. L. N. G. (2020). Do we have the guts to go? The abdominal compartment, intra-abdominal hypertension, the human microbiome and exploration class space missions. Canadian Journal of Surgery, 63(6), E581. https://doi.org/10.1503/CJS019219
- Pavlakou, P., Dounousi, E., Roumeliotis, S., Eleftheriadis, T., & Liakopoulos, V. (2018). Oxidative Stress and the Kidney in the Space Environment. International Journal of Molecular Sciences, 19(10). https://doi.org/10.3390/IJMS19103176
- Mehta, S. K., Szpara, M. L., Rooney, B. V., Diak, D. M., Shipley, M. M., Renner, D. W., Krieger, S. S., Nelman-Gonzalez, M. A., Zwart, S. R., Smith, S. M., & Crucian, B. E. (2022). Dermatitis during Spaceflight Associated with HSV-1 Reactivation. Viruses, 14(4). https://doi.org/10.3390/v14040789
- Radstake, W. E., Baselet, B., Baatout, S., & Verslegers, M. (2022). Spaceflight Stressors and Skin Health. Biomedicines 2022, Vol. 10, Page 364, 10(2), 364. https://doi.org/10.3390/BIOMEDICINES10020364
- Crucian, B. E., Makedonas, G., Sams, C. F., Pierson, D. L., Simpson, R., Stowe, R. P., Smith, S. M., Zwart, S. R., Krieger, S. S., Rooney, B., Douglas, G., Downs, M., Nelman-Gonzalez, M., Williams, T. J., & Mehta, S. (2020). Countermeasures-based Improvements in Stress, Immune System Dysregulation and Latent Herpesvirus Reactivation onboard the International Space Station – Relevance for Deep Space Missions and Terrestrial Medicine. Neuroscience & Biobehavioral Reviews, 115, 68–76. https://doi.org/10.1016/J.NEUBIOREV.2020.05.007